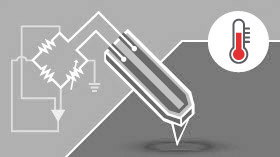
高空间分辨率和高热分辨率显微技术
我们的SThM模式允许您通过使用微加工探针测量针尖和样品之间的热传递来轻松找到样品的局部热导率。
我们的SThM模式允许您通过使用微加工探针测量针尖和样品之间的热传递来轻松找到样品的局部热导率。
人们对具有纳米结构特征的器件和材料的热特性越来越感兴趣。扫描热显微镜(SThM)被开发出来以探测纳米级的热性能。SThM使用纳米加工的热探针来实现了高空间分辨率和热分辨率以及灵敏度,并具有创新的信号检测方案。SThM有两种模式:温度对比模式(TCM)和热导率对比模式(CCM)。TCM允许用户测量带有热源的样品表面上的温度变化。CCM允许用户测量样品表面上的热导率变化。图1显示了SThM的实验装置。SThM的主要部分是SThM探针,其针尖末端具有电阻元件,以及惠斯通电桥。针尖和样品表面之间的距离由接触模式AFM反馈控制,形貌信息直接从悬臂偏转中测量,这与样品的热性能无关。在扫描形貌的同时,可以收集热CCM或TCM图像。在这里,惠斯通电桥的输出电压用作反馈,以保持探针温度恒定(CCM)或测量探针的温度变化(TCM)。
图1. . SThM实验装置示意图,展示了连接到惠斯通电桥的纳米热SThM探针。
图2. SThM纳米热探针的扫描电子显微镜(SEM)图像
SThM的关键部分是SThM针尖,它在扫描样品表面时作为电阻温度计(或在CCM中作为加热器)。惠斯通电桥电路根据表面的温度控制SThM针尖的电阻率,并在扫描的每个像素处获取电阻率。以前的基于导线的热探针(如沃拉斯顿线)由于导线几何形状的限制,无法提供足够的空间分辨率和热分辨率。改进的SThM使用纳米加工的热探针,其中电阻元件通过光刻图案化到AFM针尖上。图2显示了这种纳米加工热探针的扫描电子显微镜(SEM)图像,其针尖半径约为100纳米,能够实现高分辨率的热图像扫描。使用纳米加工的热探针,SThM同时支持TCM和CCM模式。
在热探针显微镜(TCM)中,纳米热探针的电阻元件被用作电阻温度计。当探针扫描样品表面时,热探针的温度会根据表面温度发生变化。这种探针温度的变化导致其电阻发生变化。因此,通过在探针中运行恒定电流(称为“探针电流”),可以连续测量探针电阻,从而测量探针下方样品表面的局部温度,如图3(a)所示。在扫描过程中,桥电路中的可变电阻器控制电流,以便在扫描期间只有很小的电流流经探针。由于自加热引起的电阻变化可能导致温度测量误差,因此在TCM中通过探针的电流必须足够小,以防止探针的自加热。在扫描过程中,探针电阻的变化改变了惠斯通电桥的电压平衡,引入了一个称为“SThM误差”的V中的误差信号。这种由于探针电阻变化引起的SThM误差用于在TCM中生成SThM图像,并转化为样品的定性温度分布。
图3.. TCM模式示意图,其中SThM针尖作为电阻温度计来检测温度变化
样品(a)和CCM模式(b),其中SThM针尖作为电阻加热器,并通过额外的热反馈保持恒定(温度)。随着热量从针尖流向样品,针尖温度会发生变化。
在CCM中,纳米热探针的电阻元件用作电阻加热器。向探针针尖施加足够高的电流,通过额外的热反馈回路将其保持在设定温度。维持设定温度所需的能量代表局部热导率。当样品的热导率高时,热量从针尖流向样品的速度快,系统由于大量散热而需要更多能量来维持恒定温度。CCM的示意图如图3(b)所示。在CCM模式中,探针温度必须高于样品温度,以确保热量仅从探针流向样品,导致探针散热。热反馈将此散热作为针尖电阻的变化来感知,相应地平衡桥电压,并恢复探针的电阻。样品的热导率与从针尖流向样品的热量成正比。因此,检测到的热量流动变化可用于成像样品热导率的变化。
图4.. AFM高度(a)和代表热导率的探针电流,
(b)使用纳米热探针的SThM中的CCM模式在PS/LDPE表面上进行了测量。
SThM可用于基于热导率区分聚合物复合样品中的材料变化,如图4所示。这里,通过SThM表征了硅基底上的无定形(无规立构)聚苯乙烯(PS)和低密度聚乙烯(LDPE)的混合物。LDPE是一种由嵌入无定形PS中的结晶薄片组成的半结晶聚合物。使用CCM模式,在没有额外热源的情况下测量了PS/LDPE的形貌(图4(a))和定性热导率图像(图4(b))。在图4(b)的探针电流图像中,可以清晰地区分出具有较低热导率的PS和具有较高热导率的LDPE之间的材料差异。图5(见下一页)显示了硬盘驱动器(HDD)磁头极尖回缩(PTR)的TCM测量,以研究在写入器或读取器上施加功率时的样品加热情况。在图5(a)的形貌图中标记了写入器(区域A)和读取器(区域B)。图5(b)中的SThM误差图像代表了样品上未施加功率时的初始定性表面温度分布,呈现出均匀的温度分布。然而,当在图5(c)中的写入器或在图5(d)中的读取器上施加功率时,相应区域的表面温度相比周围区域升高。因此,SThM可以可视化由于向写入器或读取器施加功率而引起的表面温度升高。由于SThM误差与针尖温度之间存在线性相关性,因此可以通过与温度控制台(TCS)进行校准,将SThM误差转换为绝对温度值。随着TCS温度的升高,SThM针尖检测到SThM误差,如图6(a)所示,并使用线性拟合方程从温度与SThM误差图中计算校准因子。校准后,可应用校准因子来计算未知温度样品上的表面温度,如图6(c)所示。
图5.. 硬盘驱动器(HDD)磁头极尖回缩(PTR)上的AFM高度(a),未向样品施加功率时的SThM图像(b),
(c)向写入器(区域A)施加功率时的SThM图像,以及(d)向读取器(区域B)施加功率时的SThM图像。
(d)显示了相应区域的温度升高。
图6. .使用温度控制台(TCS)将SThM误差转换为绝对温度值的校准过程(a),
(b)金属加热器上的AFM高度图像,以及(c)根据SThM误差计算出的金属加热器表面温度。